High-temperature superconductivity
In 1986, J. Georg Bednorz and K. Alex Mueller discovered superconductivity in a lanthanum-based cuprate perovskite material, which had a transition temperature of 35 K (Nobel Prize in Physics, 1987) and was the first of the high-temperature superconductors.
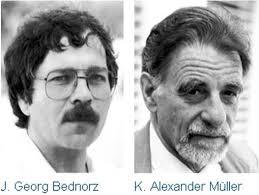
Whereas "ordinary" or metallic superconductors usually have transition temperatures (temperatures below which they are superconductive) below 30 K (−243.2 °C) and must be cooled using liquid helium in order to achieve superconductivity, HTS have been observed with transition temperatures as high as 138 K (−135 °C), and can be cooled to superconductivity using liquid nitrogen. It was shortly found (by Ching-Wu Chu) that replacing the lanthanum with yttrium, i.e. making YBCO, raised the critical temperature to 92 K, which was important because liquid nitrogen could then be used as a refrigerant (at atmospheric pressure, the boiling point of nitrogen is 77 K). This is important commercially because liquid nitrogen can be produced cheaply on-site with no raw materials, and is not prone to some of the problems (solid air plugs, etc.) of helium in piping. Many other cuprate superconductors have since been discovered, and the theory of superconductivity in these materials is one of the major outstanding challenges of theoretical condensed-matter physics.
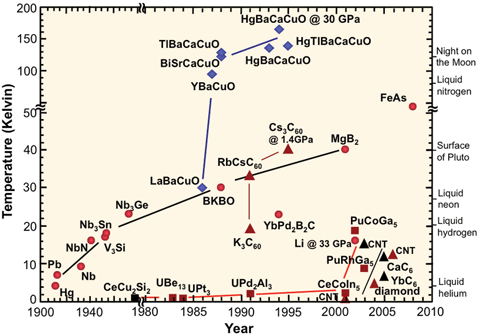
In March 2001, superconductivity of magnesium diboride (MgB2) was found with Tc = 39 K.
In 2008, the oxypnictide or iron-based superconductors were discovered, which led to a flurry of work in the hope that studying them would provide a theory of the cuprate superconductors.
In 2013, room-temperature superconductivity was attained in YBCO for picoseconds, using short pulses of infrared laser light to deform the material's crystal structure.
In 2017 it was suggested that undiscovered superhard materials (e.g. critically doped beta-titanium Au) might be a candidate for a new superconductor with Tc substantially higher than HgBaCuO (138K) possibly up to 233K which would be higher even than H2S. A lot of research suggests that additionally nickel could replace copper in some perovskites, offering another route to room temperature. Li+ doped materials can also be used i.e. the spinel battery material LiTi2Ox and the lattice pressure can increase Tc to over 13.8K Also LiHx has been theorized to metallise at a substantially lower pressure than H and could be a candidate for a Type 1 superconductor.
These high-temperature superconductors are ceramics. They contain lanthanum, yttrium, or another of the rare-earth elements or bismuth or thallium; usually barium or strontium (both alkaline-earth elements); copper; and oxygen. Other atomic species can sometimes be introduced by chemical substitution while retaining the high-Tc properties.
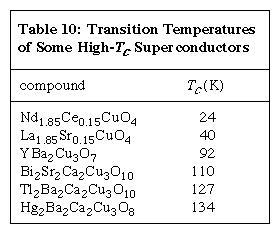
The table lists the member of each major family of high-Tc materials with the highest observed superconducting transition temperature. The value 134 K is the highest known Tc value. Within each family of high-Tc materials, only the subscripts (i.e., stoichiometry) vary from one compound to another. Samples in the families containing bismuth or thallium always exhibit a great deal of atomic disorder, with atoms in the “wrong” crystallographic sites and with impurity phases. It is possible that such disorder is required to make these compounds thermodynamically stable.
Properties
"High-temperature" has two common definitions in the context of superconductivity:
Above the temperature of 30 K that had historically been taken as the upper limit allowed by BCS theory (1957). This is also above the 1973 record of 23 K that had lasted until copper-oxide materials were discovered in 1986.
Having a transition temperature that is a larger fraction of the Fermi temperature than for conventional superconductors such as elemental mercury or lead. This definition encompasses a wider variety of unconventional superconductors and is used in the context of theoretical models.
The label high-Tc may be reserved by some authors[citation needed] for materials with critical temperature greater than the boiling point of liquid nitrogen (77 K or −196 °C). However, a number of materials – including the original discovery and recently discovered pnictide superconductors – had critical temperatures below 77 K but are commonly referred to in publication as being in the high-Tc class.
Technological applications could benefit from both the higher critical temperature being above the boiling point of liquid nitrogen and also the higher critical magnetic field (and critical current density) at which superconductivity is destroyed. In magnet applications, the high critical magnetic field may prove more valuable than the high Tc itself. Some cuprates have an upper critical field of about 100 tesla. However, cuprate materials are brittle ceramics which are expensive to manufacture and not easily turned into wires or other useful shapes. Also, high-temperature superconductors do not form large, continuous superconducting domains, but only clusters of microdomains within which superconductivity occurs. They are therefore unsuitable for applications requiring actual superconducted currents, such as magnets for magnetic resonance spectrometers.
After two decades of intense experimental and theoretical research, with over 100,000 published papers on the subject, several common features in the properties of high-temperature superconductors have been identified. As of 2011, no widely accepted theory explains their properties. Relative to conventional superconductors, such as elemental mercury or lead that are adequately explained by the BCS theory, cuprate superconductors (and other unconventional superconductors) remain distinctive. There also has been much debate as to high-temperature superconductivity coexisting with magnetic ordering in YBCO, iron-based superconductors, several ruthenocuprates and other exotic superconductors, and the search continues for other families of materials. HTS are Type-II superconductors, which allow magnetic fields to penetrate their interior in quantized units of flux, meaning that much higher magnetic fields are required to suppress superconductivity. The layered structure also gives a directional dependence to the magnetic field response.
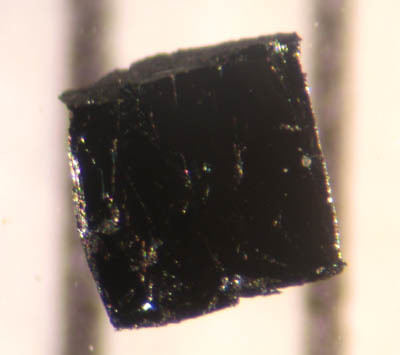
Possible mechanism
There have been two representative theories for high-temperature or unconventional superconductivity. Firstly, weak coupling theory suggests superconductivity emerges from antiferromagnetic spin fluctuations in a doped system. According to this theory, the pairing wave function of the cuprate HTS should have a dx2-y2 symmetry. Thus, determining whether the pairing wave function has d-wave symmetry is essential to test the spin fluctuation mechanism. That is, if the HTS order parameter (pairing wave function) does not have d-wave symmetry, then a pairing mechanism related to spin fluctuations can be ruled out. (Similar arguments can be made for iron-based superconductors but the different material properties allow a different pairing symmetry.) Secondly, there was the interlayer coupling model, according to which a layered structure consisting of BCS-type (s-wave symmetry) superconductors can enhance the superconductivity by itself. By introducing an additional tunnelling interaction between each layer, this model successfully explained the anisotropic symmetry of the order parameter as well as the emergence of the HTS. Thus, in order to solve this unsettled problem, there have been numerous experiments such as photoemission spectroscopy, NMR, specific heat measurements, etc. Up to date the results were ambiguous, some reports supported the d symmetry for the HTS whereas others supported the s symmetry. This muddy situation possibly originated from the indirect nature of the experimental evidence, as well as experimental issues such as sample quality, impurity scattering, twinning, etc.
This summary makes an implicit assumption: superconductive properties can be treated by mean field theory. It also fails to mention that in addition to the superconductive gap, there is a second gap, the pseudogap. The cuprate layers are insulating, and the superconductors are doped with interlayer impurities to make them metallic. The superconductive transition temperature can be maximized by varying the dopant concentration. The simplest example is La(2)CuO(4), which consist of alternating CuO(2) and LaO layers which are insulating when pure. When 8% of the La is replaced by Sr, the latter act as dopants, contributing holes to the CuO(2) layers, and making the sample metallic. The Sr impurities also act as electronic bridges, enabling interlayer coupling. With this realistic picture of the electronic and atomic structure of high temperature superconductors, one can show from thousands of experiments that the basic pairing interaction is still interaction with phonons, just as in the old metallic superconductors with Cooper pairs. While the undoped materials are antiferromagnetic, even a few % impurity dopants introduce a smaller pseudogap in the CuO2 planes which is also caused by phonons (technically charge density waves). This gap decreases with increasing charge carriers, and as it nears the superconductive gap, the latter reaches its maximum. This picture has been extended to answer the most obvious question of high temperature superconductivity, why are the transition temperatures so high? The carriers follow zig-zag percolative paths, largely in metallic domains in the CuO(2) planes, until blocked by charge density wave domain walls, where they use dopant bridges to cross over to a metallic domain of an adjacent CuO(2) plane. This model of self-organized networks of percolative paths describes all known maximum transition temperatures with no adjustable parameters. The transition temperature maxima are reached when the host lattice has weak bond-bending forces, which produce strong electron-phonon interactions at the interlayer dopants.
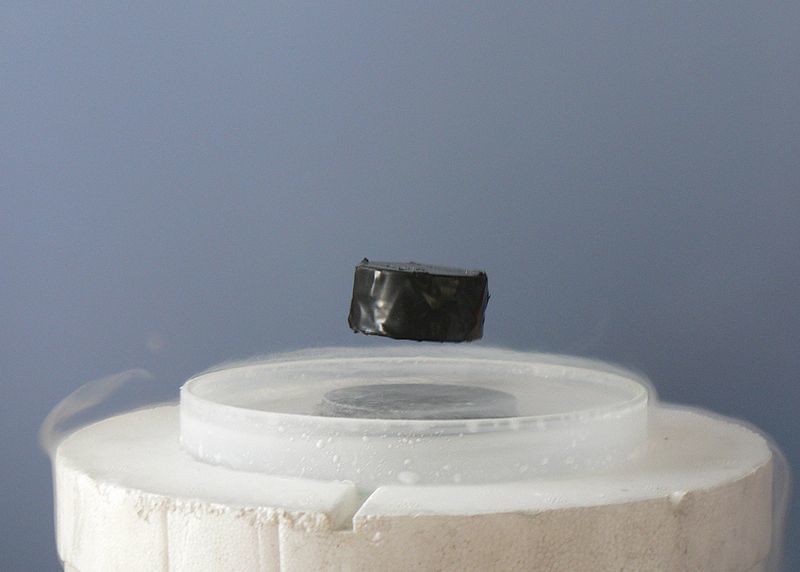





REFERENCES
Encyclopædia Britannica. Available in: https://www.britannica.com/science/superconductivity#ref912878. Access in: 28/10/2018.
The National High Magnetic Field Laboratory. Available in: https://nationalmaglab.org/news-events/feature-stories/high-temperature-superconductors. Access in: 28/10/2018.
Wikipedia. Available in: https://en.wikipedia.org/wiki/High-temperature_superconductivity. Access in: 28/10/2018.
Wikipedia. Available in: https://en.wikipedia.org/wiki/History_of_superconductivity. Access in: 28/10/2018.
0 comments
Sign in or create a free account